
What are the current obstacles to high power EUV?
In ASML's current LPP source designs, a solid state "pre-pulse" laser and a second, high energy CO2 laser are fired at micron sized tin (Sn) pellets, evaporating them and releasing EUV light as a byproduct. Knowledgeable sources have informed me that the currently employed CO2 lasers are at or near the maximum of their pulse rate capabilities, effectively limiting further power output. As more CO2 laser power becomes available, there may still be practical limitations on the scaling and feed rate of Sn (tin) target material. As determined by physics, the inherent energy conversion factor for tin approximates 4%, and further incremental improvements in efficiency are obtained with diminishing returns. Assuming additional laser power becomes available for ASML, further complications can result from higher LPP source power levels as the rate of residual particulate contamination from evaporated tin increases in approximate proportion with increased laser power. Critical beam line mirror surfaces and other source components rapidly lose their efficiencies as tin contamination accumulates, reducing the available up time of the stepper (MTBF). It was originally anticipated that an optimal LPP EUV source design would provide 13.5nm light at power levels >200 watts, providing current and future lithography requirements. However, more recent demands for even higher EUV power levels have been identified. ASML and Carl Zeiss acknowledged in an invited paper at SPIE Advanced Lithography 2015, that higher resolutions will require 60mJ/cm2 for half pitch nodes <8nm. [3] ASML's recent (2015) collaboration with Carl Zeiss has produced an optical system with a numeric aperture (NA) of 0.55 vs. ASML's current EUV NA of 0.33. The higher NA system will require 500 watts of EUV power to achieve the estimated 60 mJ/cm2 dosimetry required for throughput of 150 wafers/hour. While this concept extends the viability of 13.5nm lithography, the delivery of a reliable 500 watt EUV source remains a critical item on the agenda, meaning the availability of free electron laser technology will probably gate related programs. A recent article appearing in the SPIE News Room, Extending extreme-UV lithography technology, [4] suggests that power levels of 500 – 1000 watts may be required for a single stepper necessitating a large scale central source EUV FEL.
A Primer on Free Electron Lasers
What is a free electron laser and how is it different from conventional lasers and LPP systems? To answer this question we must entertain the convergence of the US DOE's high energy physics community with the semiconductor industry and discuss recent innovations in technology. In previous and current generation stepper and scanner systems, it's been common to utilize laser light sources producing the desired wavelengths required for semiconductor photolithography. In current 193nm lithography systems, an argon fluoride (ArF) laser produces the light. The laser light produced is monochromatic, of sufficient brilliance and provides many hours of trouble free uptime. It would seem this simplistic approach might be applied to EUV lithography. Why not build an EUV laser with a wavelength of 13.5 nanometers? This has not been possible due to limitations in physics. The highly reflective optics required for laser efficiencies have yet to be created for EUV spectra. Current Bragg cell mirrors reflect EUV with a closely approximated 90% efficiency. However, FEL is a game changer. Some history and an analogy:
The term LASER is an acronym for Light Amplification by Stimulated Emission of Radiation. The first solid state, 694nm synthetic ruby laser produced at Hughes Research in 1960, [5] utilized xenon flash lamps to inject high energy photons throughout the core of a ruby rod, stimulating the emission of photons from its lattice structures. Lasers operate at specific wavelengths which are determined by the seed (or lazing) material's inherent spectral signature. The 694nm wavelength is derived from the band gap emissions of the ruby's crystalline composition. We might compare the ruby crystal in this laser with a quartz crystal in a radio which determines its operational frequency. Accordingly, we might otherwise assign “channel I” as an identifier of I-line photolithography operating at 365nm.
The Foundation Physics of FEL
A Radio Logical Analogy
A radio transmitter's frequency has historically been controlled by quartz crystal elements. Y-cut quartz crystals oscillate (vibrate) at specific frequencies which are dependent upon their thickness. The thinner the crystal, the higher the frequency obtained. Inversely, the thicker the crystal, the lower the frequency obtained. Passing an electric current through a quartz crystal induces it to oscillate at its inherent resonant frequency, so determined by its thickness. The frequency produced is extremely stable and the resulting wave form is of high purity, providing an excellent medium for control of radio frequencies and instrumentation. Crystals also produce harmonic frequencies. A harmonic is a multiple of the crystal's fundamental resonant frequency. As such, a crystal oscillating at 3 MHz will also produce a weaker signal at 6MHz (its second harmonic frequency), and a still weaker third harmonic at 9MHz and so on. When impractical to manufacture crystals at their desired fundamental frequencies, "third overtone" crystals are often utilized to provide a harmonic frequency which can be sufficiently amplified and utilized as an effective fundamental frequency, thus extending the upper limits (and our usage) of the radio spectrum. Even with clever engineering, over the years radio frequency control became problematic as multi-channel communication systems evolved, requiring large banks of crystals to span a given range of frequencies; one crystal required for each channel frequency. Rather than utilize thousands of crystals to span the radio spectrum, communications equipment evolved to employ a frequency control device called a VFO: a Variable Frequency Oscillator. In this scenario, several fundamental frequency crystals and specially designed varactor diode/phased locked loop circuits comprise a heterodyne oscillator, sometimes known as an IF (Intermediate Frequency) mixer. Such an oscillator can generate a wide range of possible frequency combinations by mixing (heterodyning) the output obtained from the crystals to produce the desired sum/difference of their frequencies by way of constructive or destructive interference. As a VFO radio tuning dial is manipulated, it changes one of the mixing frequencies to produce the desired sum/difference operational frequency for both transmitter and receiver. The advantage to such a design is the elimination of separate transmitter/receiver controls, and thousands of individual crystals normally assigned for each desired radio channel. The conceptual use of both harmonic and sum/difference frequency synthesis has found its way into many applications in physics and electronics.
Free Electron Laser Fundamentals
Imagine that we might adjust and control a laser's wavelength using a concept similar to a radio's Variable Frequency Oscillator but with a different set of physics. By electronically tuning a laser's wavelength, we can eliminate the need for specialized crystalline, gaseous or other lazing materials and operate outside the spectral wavelength segments they are physically limited to. FEL technology can produce tunable wavelengths of light throughout the microwave, visible spectrum and x-ray regime. A free electron laser is comprised of a large beamline/electron source which accelerates electrons to near the speed of light. On opposite sides of the electron beam line are interposed field coils of opposing polarity called undulators or "wigglers", which when energized establish a transverse sinusoidal field across the beam path. Electrons accelerated into the transverse field produce incoherent photons in a mixed assortment of sinusoidal wavelengths sometimes referred to as “bunches”, emitting photons at wavelengths determined by their acceleration and the transverse field strength (synchrotron radiation). By adjusting the electron beam energy or the magnetic field strength of the undulators, the wavelength of the emitted photons can be tuned selectively to produce coherent light. Variations on this concept have evolved as follows:
A Tunable SASE FEL
A SASE FEL is able to produce laser light over a broad range of spectrum without the requirement for conventional lazing materials such as ruby crystal or argon fluoride etc. In a tunable SASE (Self Amplified Spontaneous Emission) FEL, high energy source electrons passing through an undulator can produce an assortment of incoherent photons (initially at randomly different wavelengths) which become bunched in the transverse sine wave and interact via constructive or destructive interference, producing incidental derivative wavelengths (spontaneous emission). That is to say the bunched photons add and subtract their wavelength values from one another producing new sum/difference valued photons at the mathematically resulting wavelengths. When tuned to a specific wavelength of interest by adjusting the electron beam energy or the magnetic field strength of the undulators, such subsequently produced photons arrive in phase (at the same wavelength) and cumulatively intensify to release high energy coherent laser light (self amplification). While a very useful concept for a variety of applications, the spontaneous emission in a SASE FEL can propagate statistical artifacts resulting from the inherent mathematical sum/difference phenomenon, and consequently can produce a beam exhibiting limited shot to shot reproducibility. As such, the utility of a SASE FEL might be limited in applications which require extremely accurate dosimetry. The limited shot to shot reproducibility might also contribute to the dosimetry phenomenon known as “shot noise”.
A Tunable HGHG FEL
FEL performance can be modified and improved by utilizing an external seed laser as a source wavelength. The seed laser is a conventional laser utilizing a material such as ruby crystal (one example) to produce a monochromatic feed source of photons. In an HGHG (High Gain Harmonic Generation) FEL, the seed laser interacts with the electron beam as it propagates through the first undulator (called a modulator), tuned to the seed laser's wavelength. The resulting interaction with the seed laser induces coherent modulation of the electron beam energy, creating photon bunching as well as consequential harmonic propagation (photons which are the mathematical multiples of the seed laser's wavelength). The micro-bunched beam of photons are then injected into a long undulator tuned to the desired harmonic wavelength. The desired wavelength comprised of harmonically produced photons arrive in phase and cumulatively intensify to release high energy coherent light at the newer, shorter wavelength of interest. A recent FERMI paper illustrates 500 shot reproducibility of 8th harmonic spectra at 32.5nm (obtained from a 260nm seed laser) exhibiting normalized photon/energy stability in the order of 7x10^-5 (root mean square), a marked improvement over previous SASE FEL data obtained over the same photon energy range. The high purity monochromatic spectra of an HGHG seed laser improves the system's shot to shot repeatability as its mode of operation does not incur the statistical deviation phenomena found in spontaneous emission spectra typically observed in a SASE FEL. As such, an HGHG FEL might be more advantageous for use in EUV applications requiring highly precise dosimetry, possibly reducing shot noise phenomenon.
The EUV Source Challenge Ahead
Large scale projects are underway to build FEL systems to accommodate a wide range of wavelengths and scientific applications. FEL is next generation laser technology which is perhaps the best candidate to replace the LPP/EUV source designs currently offered by ASML.
Known for its work in actinic inspection at the 13.5 nm EUV wavelength, Lawrence Berkeley CXRO Lab [6] is also part of a DOE consortium currently working on LCLS-II (Linac Coherent Light Source-II) [7] at Lawrence Livermore and SLAC. Last June at the 2015 International Workshop on EUV Lithography in Maui, Aaron Tremaine of SLAC presented a comprehensive review of possible FEL designs that might be considered for EUV lithography and identified the consortium of DOE laboratories participating in the EUV FEL program. The Who's Who list of DOE participants includes SLAC, Lawrence Berkeley National Labs, Fermilab, Argonne National Lab, Cornell, UCLA, RadiaBeam, AES and Radiasoft. In order to appreciate the scale and capital intensity of this project it becomes necessary to review Aaron Tremaine's 2015 EUV Litho, Inc. Workshop presentation, LCLS-II and Free electron laser drivers for EUV Lithography [8]. The report describes FEL design considerations and recommends a “Straight Shooter” beamline configuration for semiconductor EUV lithography applications. The report addresses Erik R. Hosler's (GLOBALFOUNDRIES) 2015 SPIE publication, “Considerations for a free-electron laser based extreme-ultraviolet lithography program”, (Proc. of SPIE Vol. 9422, 94220D, 2015). The good news is that many new FEL programs are in progress [9] and the LCLS-II at SLAC might provide a viable, solution for HVM/EUV lithography. More recently, visible collaboration between GLOBALFOUNDRIES and SLAC has established the ground work for possible in-fab FEL source designs. The subsequent challenge for any future FEL/EUV initiative, is that once again the convergence of the semiconductor industry and our national laboratory community will be required to deliver future lithography source technology for EUV and beyond.
ASML has taken the lead in providing viable interim EUV technology permitting the characterization of materials, resists, masks and process precision required for future generation lithography. Double patterning techniques utilizing 193i lithography will continue to enable CDs =<10nm. We can also speculate how 13.5nm multiple patterning might enable future nodes and continued process development. In the interim, the current ASML LPP/EUV initiative has enabled the ground work our industry requires for future precision nanometer scale lithography.
Let's continue working together to secure next generation EUV and the preservation of Moore's Law.
During the course of researching this article I digested many components of the SLAC Conceptual Design Report for the LCLS-II (Linear Coherent Light Source). Among many, two components of the LCLS-II are the SXR (Soft X-Ray) and HXR (Hard X-Ray) undulators and their respective beam lines. It is with some amusement that my FCC designated amateur radio call sign is WA2HXR which I acquired in 1970. It should be noted that my amateur radio operations are restricted to applicable licensed amateur radio frequency spectra which excludes X-Ray wavelengths.
CQ SLAC CQ SLAC CQ SLAC DE WA2HXR K. 73
Click here to download this article.
Thomas D. Jay
Semiconductor Industry Consultant
Thomas.Dale.Jay@gmail.com
https://ThomasDaleJay.blogspot.com
Thomas D. Jay YouTube Channel
Visit my new Amateur Radio blog at:
www.WA2HXR.blogspot.com
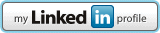


Corporate, private entities or publications referenced or linked in this article are the respective owners of their logos, trademarks, service marks, media content and intellectual property. Unless otherwise disclosed, Thomas D. Jay has no financial interest in companies referenced in blog articles or other published media communications. Thomas D. Jay is not a registered financial advisor. No representation is made to either buy or sell securities. Opinions expressed by Thomas D. Jay are his own. Thomas D. Jay does not employ or otherwise utilize/authorize third party agents to express his opinions, represent his interests or conduct business on his behalf except where formally contractually designated. Thomas D. Jay does not agree to indemnify or hold harmless vendors, clients or third parties to related contractual agreements and reserves the right to applicable legal remedies in lieu of arbitration. These terms are subject to change. Concerned parties should check this blog site for periodic updates.
Acknowledgments and Reference Links
[1] Free Electron Laser
Wikipedia
[2] Future FEL/EUV Strategy – The Light at the End of the Beam Line
Thomas D. Jay, Blog Publication, September 20, 2014
[3] ASML and Carl Zeiss acknowledged in an invited paper at SPIE Advanced Lithography 2015, that higher resolutions will require 60mJ/cm2 for half pitch nodes <8nm
SPIE Proceedings
[4] Extending extreme-UV lithography technology
SPIE News Room, Erik R. Hosler, Obert R. Wood II, Moshe E Preil
[5] The first solid state, 694nm synthetic ruby laser produced at Hughes Research in 1960
Wikipedia
[6] Lawrence Berkeley CXRO Lab
Lawrence Berkeley National Labs CXRO Web Site
[7] Linac Coherent Light Source-II
Stanford Linear Accelerator Web Site
[8] LCLS-II and Free electron laser drivers for EUV Lithography
Aaron Tremaine Presentation, SLAC
EUV Litho, Inc. Web Site, 2015 Program, Maui
[9] many new FEL programs are in progress
University of California, Santa Barbara FEL Web Site
Site Maintained by G. Ramian
Visit my new Amateur Radio blog at:
www.WA2HXR.blogspot.com
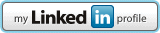


Corporate, private entities or publications referenced or linked in this article are the respective owners of their logos, trademarks, service marks, media content and intellectual property. Unless otherwise disclosed, Thomas D. Jay has no financial interest in companies referenced in blog articles or other published media communications. Thomas D. Jay is not a registered financial advisor. No representation is made to either buy or sell securities. Opinions expressed by Thomas D. Jay are his own. Thomas D. Jay does not employ or otherwise utilize/authorize third party agents to express his opinions, represent his interests or conduct business on his behalf except where formally contractually designated. Thomas D. Jay does not agree to indemnify or hold harmless vendors, clients or third parties to related contractual agreements and reserves the right to applicable legal remedies in lieu of arbitration. These terms are subject to change. Concerned parties should check this blog site for periodic updates.
Acknowledgments and Reference Links
[1] Free Electron Laser
Wikipedia
[2] Future FEL/EUV Strategy – The Light at the End of the Beam Line
Thomas D. Jay, Blog Publication, September 20, 2014
[3] ASML and Carl Zeiss acknowledged in an invited paper at SPIE Advanced Lithography 2015, that higher resolutions will require 60mJ/cm2 for half pitch nodes <8nm
SPIE Proceedings
[4] Extending extreme-UV lithography technology
SPIE News Room, Erik R. Hosler, Obert R. Wood II, Moshe E Preil
[5] The first solid state, 694nm synthetic ruby laser produced at Hughes Research in 1960
Wikipedia
[6] Lawrence Berkeley CXRO Lab
Lawrence Berkeley National Labs CXRO Web Site
[7] Linac Coherent Light Source-II
Stanford Linear Accelerator Web Site
[8] LCLS-II and Free electron laser drivers for EUV Lithography
Aaron Tremaine Presentation, SLAC
EUV Litho, Inc. Web Site, 2015 Program, Maui
[9] many new FEL programs are in progress
University of California, Santa Barbara FEL Web Site
Site Maintained by G. Ramian